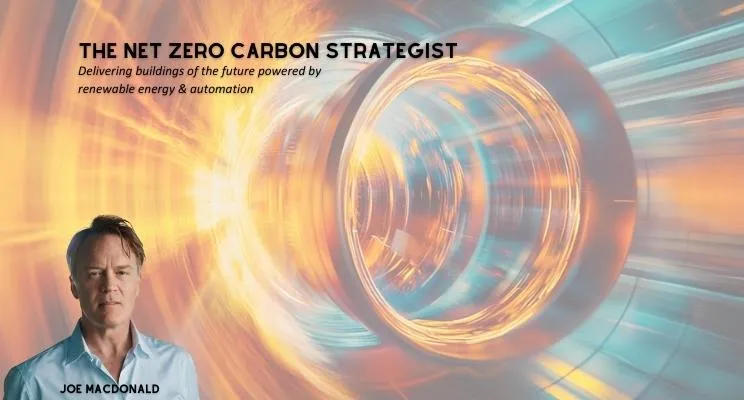
Compressed Air Energy Storage (CAES): A Comprehensive 2025 Overview
1. Introduction
Compressed Air Energy Storage (CAES) has emerged as one of the most promising large-scale energy storage technologies for balancing electricity supply and demand in modern power grids. Renewable energy sources such as wind and solar power, despite their many benefits, are inherently intermittent. On windy days or during periods of bright sunlight, the supply of renewable energy can exceed demand, while at other times, renewable generation may fall short of consumption needs. CAES offers a powerful means to store excess electricity by using it to compress air, which can be released and expanded through a turbine to generate electricity when the grid requires additional power.
First proposed in the mid-20th century, CAES technology has gained renewed attention in the 21st century due to the global push for decarbonization and the rapid expansion of renewable energy capacity. By storing vast amounts of energy in geological formations, depleted gas reservoirs, or even specially designed vessels, CAES systems can provide gigawatt-scale storage over extended durations—from hours to days or even months in certain contexts. This makes them uniquely suited to address the large-scale, long-duration storage challenge posed by renewable power generation.
Beyond renewable integration, CAES also benefits grids by providing ancillary services, improving power quality, and even facilitating “black start” operations to recover from major grid outages. While the technology’s round-trip efficiency traditionally lags behind that of batteries, ongoing research—especially in adiabatic CAES (A-CAES)—has substantially improved system performance and reduced dependence on fossil fuels.
In this article, we explore the principles of CAES, its historical development, critical infrastructure requirements, various system configurations, benefits, challenges, current global deployments, and the future trajectory of this technology. We will also touch on new horizons for CAES, including data center applications, eco-resorts, and how artificial intelligence (AI) is poised to enhance its performance and operational efficiency.
2. Historical Development and Context
The concept of storing energy through the compression of air dates back to at least the late 19th century, but large-scale implementations began taking shape only in the 20th century. Early research into CAES paralleled developments in gas turbine technology, as engineers realized that compressed air could significantly reduce the startup time and improve the flexibility of gas-fired power plants.
The first commercial CAES plant came online in Huntorf, Germany, in 1978. At a capacity of around 290 MW, it was a pioneering project that showcased the viability of storing and then re-expanding compressed air for electricity generation. The Huntorf plant used salt caverns to store pressurized air and employed a diabatic process—meaning the heat from compression was not stored but instead vented to the atmosphere. To reheat the expanding air, the system combusted natural gas.
In 1991, the McIntosh plant in Alabama, USA, became the second commercial CAES facility. It introduced several technological improvements over Huntorf, including better thermal management and efficiency. Despite these advancements, both plants still used natural gas to heat the compressed air prior to expansion, reflecting the diabatic CAES approach.
Throughout the 1990s and early 2000s, various demonstration projects and research initiatives explored how to improve CAES efficiency and reduce its carbon footprint. These efforts led to the conception of adiabatic CAES (A-CAES), in which the heat generated during compression is stored separately and later reused to heat the air during expansion.
By 2012, with the Gaines, Texas, project (500 MW capacity) and other pilot programs, the idea of CAES as a large-scale, long-duration energy storage solution gained traction. Today, increasing interest in renewable integration, decarbonization policies, and the maturity of geological surveying techniques have led to renewed efforts to refine CAES and explore more sites for potential deployment worldwide.
3. Fundamental Principles of CAES
CAES is conceptually straightforward yet technologically intricate in practice. The fundamental process includes three primary stages:
1. Compression: When there is surplus electricity—often during periods of low demand or high renewable generation—this excess power drives electric compressors to pressurize ambient air. This compressed air is then channeled into a dedicated storage chamber.
2. Storage: The compressed air is stored, typically in large underground caverns such as salt domes, abandoned mines, or depleted natural gas reservoirs. Above-ground alternatives include high-pressure tanks or specially designed vessels, though these are generally more expensive and limited in capacity. The storage medium must be structurally capable of handling large volumes of air at high pressure while maintaining integrity over years or decades of operation.
3. Generation: During peak demand or when electricity prices are high, the compressed air is released from storage. In diabatic systems, the air is heated with an external heat source (often natural gas) before expansion through a turbine connected to a generator. In adiabatic systems, the heat removed during compression is stored and then re-injected into the air stream prior to expansion, eliminating or drastically reducing the need for external fuels.
The thermodynamics of CAES revolve around the interplay of air pressure, temperature, and volume. By capturing the thermal energy produced during the compression stage, advanced CAES configurations can significantly boost efficiency. However, traditional (diabatic) systems vent much of this heat, leading to lower round-trip efficiency (typically between 40% and 60%).
From a grid operator’s perspective, CAES can offer high-capacity storage that can be discharged for extended periods, ranging from several hours to potentially weeks or months, depending on the site’s geological constraints and system design. This capability allows CAES not just to cover daily peak loads but also to address longer-term supply-demand imbalances—vital when integrating large proportions of wind or solar.
Moreover, because the compressor and turbine can be sized independently, CAES systems provide operational flexibility. A facility can compress air whenever surplus energy is available (or even provide ancillary services such as frequency regulation), while dispatching power as needed to meet grid demand.
4. Types of CAES
4.1 Diabatic CAES
Diabatic CAES is the traditional and historically most common form of this technology. In a diabatic system, no effort is made to store the heat generated during the compression phase. Instead, it is dissipated into the environment, typically through intercoolers or aftercoolers that protect compression equipment. As a result, when the air is released from the cavern for power generation, it must be reheated to prevent damage to turbines. This reheating is commonly done by burning natural gas.
• Efficiency and Fuel Use: Diabatic CAES systems usually exhibit round-trip efficiencies ranging from about 40% to 60%. The need for natural gas (or another fuel) increases operating costs and adds to carbon emissions.
• Examples: The pioneering Huntorf plant in Germany (operational since 1978) and the McIntosh plant in Alabama, USA (since 1991), both employ diabatic processes.
4.2 Adiabatic CAES (A-CAES)
Adiabatic CAES is a more advanced and environmentally friendly approach. During compression, significant heat is generated. Instead of venting this heat, A-CAES systems capture and store it in a thermal energy storage (TES) medium—such as molten salt, pressurized water, or specialized ceramic materials. When the compressed air is later discharged, the stored heat is fed back into the airflow before it enters the turbine.
• Efficiency Gains: By reusing the compression heat, A-CAES eliminates or minimizes the need for external fuels during expansion, which substantially improves the system’s round-trip efficiency, theoretically exceeding 70% (some designs even aim for 80%+).
• Environmental Benefits: A-CAES greatly reduces or eliminates greenhouse gas emissions associated with fuel combustion. If powered by renewables, the entire cycle can be virtually carbon-free.
• Challenges: Storing large quantities of heat at high temperatures is technically challenging, requiring advanced insulation, thermal fluids, and robust materials to handle thermal stress.
4.3 Isothermal and Near-Isothermal CAES
While less commonly implemented at large scales, isothermal and near-isothermal CAES aim to maintain a nearly constant temperature during compression and expansion. This is achieved by orchestrating slow compression and expansion processes or by employing heat exchangers that continuously remove or add heat to the air stream.
• Efficiency Potential: In an ideal isothermal process, the theoretical efficiency can approach 100%, since there is minimal heat loss. However, achieving true isothermal behavior in practice is extremely difficult, especially at large power ratings.
• Practical Implementations: Near-isothermal CAES systems often use advanced heat exchange techniques or water sprays inside the compressor. Though highly efficient in small-scale prototypes, scaling these systems to utility levels remains a technical hurdle.
Each of these CAES configurations offers distinct trade-offs in terms of capital costs, efficiency, environmental impact, and complexity. While diabatic CAES remains the most prevalent commercially, adiabatic approaches are gaining ground as research and pilot projects demonstrate their feasibility and potential for zero-emission operation.
5. Key Components and Infrastructure
A CAES facility typically encompasses three major subsystems—compression, storage, and expansion. Each subsystem requires specialized engineering to ensure safe, efficient, and reliable operation:
5.1 Compression Stage
• Compressors: Industrial-scale compressors (often multi-stage, with intercooling) are used to pressurize the air. These machines can be driven by electric motors using surplus grid power or dedicated on-site generation.
• Intercoolers and Aftercoolers: In diabatic systems, these coolers dissipate heat to protect the compressor stages. In adiabatic or near-isothermal systems, a portion or all of this heat is captured and stored in a thermal energy storage unit.
5.2 Storage Stage
• Underground Caverns: Salt domes, depleted oil or gas fields, and aquifers are commonly used for large-scale CAES. The geological formation must be airtight, structurally stable, and located at a suitable depth. Salt caverns are particularly favored due to their low permeability and relative ease of solution mining.
• Above-Ground Vessels: For smaller-scale applications or regions lacking suitable geology, high-pressure steel tanks or specially engineered vessels can store compressed air. While more flexible in siting, these options typically have smaller capacity and higher material costs.
5.3 Expansion and Power Generation Stage
• Turbines: The compressed air is released through expansion turbines to generate electricity. In diabatic systems, natural gas combustion chambers may reheat the air; in adiabatic systems, the stored thermal energy is injected to maintain high temperatures.
• Generators: Coupled to the expansion turbines, these generators deliver power back to the grid. CAES plants may also have the ability to provide ancillary services such as reactive power support, frequency regulation, and spinning reserve.
Designing each subsystem requires detailed thermodynamic modeling, safety studies, and economic considerations. Proper integration and control among these components is critical for achieving high efficiency, safe operations, and long equipment lifespans.
6. Advantages of CAES
6.1 Large-Scale Storage
CAES stands out for its ability to store substantial amounts of energy, ranging from a few megawatts to multiple gigawatts. While battery storage has grown rapidly, utility-scale battery farms rarely reach the multi-gigawatt-hour capacity that CAES can offer, especially for long-duration requirements.
6.2 Long Storage Duration
Because CAES facilities rely on large storage caverns with minimal leakage (especially in salt domes) and low self-discharge, they can store compressed air for extended periods—months or even longer. This makes CAES well-suited for seasonal storage or bridging multi-day lulls in renewable generation, in contrast to most electrochemical batteries, which are typically optimized for shorter durations (minutes to a few hours).
6.3 Environmental Friendliness
When powered by surplus renewable energy, CAES can be a low-carbon or even zero-carbon storage method (particularly in adiabatic systems where no fossil fuel combustion is necessary). It also avoids the use of hazardous chemicals and the extensive mining of metals often associated with battery production. Additionally, CAES reduces the need for peaker plants, many of which are fossil-fueled, further contributing to emission reductions.
6.4 Cost-Effectiveness
At scale, CAES can be cost-competitive due to relatively low capital costs per stored kilowatt-hour, especially if suitable geological formations are available. The levelized cost of storage often drops substantially as project size increases. Additionally, CAES systems have long operational lifespans (potentially 30 years or more) with less degradation than batteries, which require periodic replacement.
6.5 Flexibility and Grid Services
CAES provides operational flexibility by allowing independent sizing of the compressor and turbine, enabling it to act as both a flexible load and a generation source. This is particularly beneficial in grids with a high share of renewables, where ramping services and fast-responding reserves are crucial. Moreover, CAES can deliver ancillary services, including black start capability, frequency regulation, and voltage support.
In summary, CAES’s high capacity, extended duration, and comparatively favorable environmental profile distinguish it among large-scale energy storage solutions. Although installation costs and site constraints can be significant, the long-term value provided by CAES in terms of grid reliability, renewable integration, and cost savings has made it an increasingly attractive technology for utilities and investors worldwide.
7. Challenges and Limitations
Despite its numerous advantages, CAES faces certain technical, economic, and regulatory hurdles that can limit widespread adoption:
7.1 Efficiency Concerns
Traditional diabatic CAES systems can have round-trip efficiencies in the 40-60% range, primarily due to the thermal energy lost during compression. Although advanced adiabatic systems can theoretically reach efficiencies of 70-80%, developing robust thermal energy storage and heat management solutions remains a challenge. Even with adiabatic designs, system losses in compressors, expanders, and piping can reduce the net efficiency below theoretical maxima.
7.2 Geographic Constraints
CAES projects that rely on large underground caverns require specific geological formations such as salt domes or depleted gas fields. Such formations must be geologically stable, adequately sealed, and located at depths and thicknesses suitable for storage. As a result, not all regions are suitable for large-scale CAES. Above-ground storage solutions exist but are often more expensive and limited in capacity.
7.3 Heat Management
Adiabatic CAES depends on capturing and storing large amounts of heat from the compression process, which can reach temperatures over 500°C. Storing this heat efficiently and then reintroducing it into the expanding air requires advanced materials and heat-exchange systems. Any inefficiencies in thermal energy storage directly impact overall round-trip efficiency.
7.4 Capital Costs and Maintenance
While CAES can be cost-effective for large-scale storage, the initial capital expenditure can be high, especially for drilling and engineering large salt caverns or refurbishing depleted reservoirs. Additionally, CAES involves rotating machinery (compressors and turbines), which can incur higher maintenance costs compared to solid-state storage (e.g., batteries). However, this is often offset by the longer operational lifespan of CAES infrastructure.
7.5 Regulatory and Standardization Hurdles
In many regions, frameworks for permitting underground gas storage or large-scale energy projects may not be fully adapted to CAES. Regulatory bodies must ensure that geological risks (such as potential subsidence or leakage) are mitigated, and environmental impact assessments are satisfied. Lack of clear guidelines and standardized performance metrics can slow project approvals and investment.
These challenges underscore the importance of continued research, development, and demonstration projects that focus on improving efficiency, exploring new geological options (or above-ground alternatives), and clarifying regulatory pathways. As the global energy sector advances toward decarbonization, addressing these hurdles will be critical for unlocking CAES’s full potential.
8. Comparisons with Other Energy Storage Technologies
8.1 CAES vs. Batteries
Efficiency: Lithium-ion batteries generally have a higher round-trip efficiency (85-95%) than CAES, which can average around 60-70% in advanced designs.
Scale and Duration: CAES excels at very large storage capacities and multi-hour to multi-day durations, while batteries are typically more economical for shorter durations (a few hours or less).
Cost: For massive, long-duration projects, CAES can achieve a lower levelized cost of storage than lithium-ion systems. Batteries, however, remain more cost-competitive in short-duration, distributed applications.
Environmental Impact: CAES avoids some of the raw material and end-of-life disposal challenges associated with battery chemistries. Lithium-ion batteries rely on materials like lithium, nickel, or cobalt, which have their own environmental footprints.
8.2 CAES vs. Pumped Hydro Storage
Geographical Dependence: Both CAES and pumped hydro rely on favorable geology, though pumped hydro specifically needs large elevation differences and available water. CAES typically requires underground caverns or reservoirs, but it can also use above-ground vessels at smaller scales.
Scale: Pumped hydro is the world’s most widely used large-scale energy storage technology. It can exceed gigawatt capacities. CAES also can reach gigawatt scale but remains less common globally.
Environmental Impact: Pumped hydro can significantly impact local ecosystems due to damming, water diversion, and flooding of valleys. CAES generally has a smaller surface footprint, especially if using existing geological cavities.
Round-Trip Efficiency: Pumped hydro typically achieves 70-85% efficiency, higher than older diabatic CAES but comparable or slightly lower than advanced A-CAES.
8.3 CAES vs. Hydrogen Storage
Conversion Process: Hydrogen storage involves using excess electricity for water electrolysis, storing hydrogen, and then re-electrifying via fuel cells or gas turbines. CAES simply compresses air and re-expands it.
Efficiency: Hydrogen-based systems can suffer from relatively low round-trip efficiency (30-50%), although improvements in electrolyzer and fuel cell technology are ongoing. CAES can achieve up to 70% in advanced designs.
Infrastructure Requirements: Hydrogen storage requires specialized tanks or underground caverns, plus additional safety measures for handling hydrogen’s flammability. CAES primarily deals with pressurized air, which is less hazardous, though high-pressure systems also require rigorous safety protocols.
Scalability: Both CAES and hydrogen can, in theory, scale to very large capacities. However, hydrogen offers the added benefit of direct use as a fuel in other sectors (e.g., transportation, industry).
In sum, CAES distinguishes itself with its ability to provide bulk, long-duration storage at relatively low costs and with fewer environmental concerns than certain battery technologies. However, its efficiency remains lower than some battery solutions, and it is geographically constrained compared to the modularity of battery systems. Each storage technology thus occupies a unique niche, and a future clean energy grid may employ multiple solutions, with CAES offering a compelling option for large-scale, long-duration applications.
9. Current Implementations and Case Studies
9.1 Huntorf Plant (Germany)
The Huntorf CAES plant in Lower Saxony, Germany, is historically significant as the world’s first commercial CAES facility. Commissioned in 1978, it has a capacity of approximately 290 MW. This diabatic plant stores compressed air in two salt caverns and uses natural gas to reheat the air during expansion. Despite a modest round-trip efficiency (around 42%), Huntorf remains operational and provides a valuable reference point for future CAES developments. Its decades-long performance has demonstrated CAES’s durability and reliability.
9.2 McIntosh Plant (United States)
Located in McIntosh, Alabama, and operational since 1991, this 110 MW diabatic CAES facility improved on some of Huntorf’s design aspects. With a higher efficiency (around 54%), McIntosh underscored the potential for incremental gains in diabatic CAES technology. The plant employs a solution-mined salt cavern for storage and uses natural gas to reheat compressed air before expansion. Over the years, it has proven a stable source of peak power and ancillary grid services for the region.
9.3 Gaines, Texas (United States)
Completed in 2012, the Gaines CAES project in Texas (500 MW) further demonstrated the viability of large-scale storage in salt formations. Although it also follows a diabatic approach, it incorporates more advanced control systems, improved compressors, and better thermal management strategies. Gaines CAES plays a role in balancing the growing wind power capacity in Texas, which often generates excess electricity during off-peak hours.
9.4 Hubei Yingchang Project (China)
One of the most notable recent developments is the Hubei Yingchang CAES project in China. With a rated power of 300 MW and 1,500 MWh (5 hours) of discharge capacity, this project focuses on large-scale, grid-connected storage to aid the integration of renewable energy. The site repurposes abandoned salt mines for air storage, reducing land use and mitigating potential geological hazards. The round-trip efficiency is estimated to be around 64-70%. This project can help store approximately 498 GWh of energy annually while delivering around 319 GWh, enabling significant peak shaving and supporting renewable integration in the region. Additionally, by reusing abandoned salt mines, the project demonstrates an eco-friendly approach to energy storage infrastructure.
The Hubei Yingchang facility also plays a crucial role in reducing regional carbon emissions. Estimates suggest it may save over 150,000 metric tons of standard coal annually, contributing to China’s broader decarbonization goals. It stands as an example of how policy support, technological advancement, and geological resources can align to bring large-scale CAES to fruition.
10. Environmental Impacts of CAES
10.1 Emissions Profile
Diabatic CAES facilities emit CO₂ and other pollutants due to the combustion of natural gas during the expansion stage. However, their total emissions remain significantly lower than those of conventional fossil fuel power plants. Advanced adiabatic CAES systems reduce or eliminate the need for combustion, thereby drastically cutting greenhouse gas emissions. When charged using renewable energy sources, adiabatic CAES can be virtually emission-free.
10.2 Land Use and Geological Considerations
Unlike pumped hydro storage, which can require large reservoirs and potentially disrupt local ecosystems, CAES primarily uses underground geological formations, limiting surface land footprint. Nonetheless, subsurface exploration and cavern solution mining must be carefully managed to avoid aquifer contamination, land subsidence, or other geological disturbances. The use of abandoned salt mines, as exemplified by the Hubei Yingchang project, can repurpose existing voids while stabilizing local geology.
10.3 Water Consumption
CAES typically requires water for cooling compressors and for processes related to solution mining in salt domes, although the volume is usually less than that used by some thermal power plants or pumped hydro storage. Careful water management strategies, such as closed-loop cooling systems, can further reduce water usage.
10.4 Decommissioning and Lifecycle Aspects
From a lifecycle perspective, CAES systems often have a long operating life of 30 years or more. Upon decommissioning, caverns can sometimes be sealed, reused, or repurposed for other forms of storage. Steel components (e.g., turbines, compressors) can be recycled, and the environmental impact of disposal is typically lower than for large battery banks, which contain toxic or non-recyclable components.
Overall, CAES compares favorably to many other energy storage technologies in terms of land use, resource extraction, and waste management, especially if adiabatic methods and environmentally responsible siting practices are employed. Its environmental profile can be highly dependent on local geology, the source of charging power, and whether fossil fuels are used for reheating. Nevertheless, in scenarios where CAES is charged by renewable energy and employs adiabatic heat storage, it can offer a near-zero-emission storage solution at scale.
11. “Black Start” Capability and Grid Stability
A distinctive feature of many CAES systems is their “black start” capability. In the event of a widespread grid outage, most conventional power plants cannot restart without an external power source to energize critical auxiliary systems (e.g., pumps, control systems, excitation for generators). CAES plants, on the other hand, can potentially use stored compressed air to drive turbines and produce electricity without relying on external grid power.
Benefits of Black Start Capability:
1. Rapid Grid Recovery: With a CAES facility available, the time to restore power after a blackout may be significantly reduced, as it can provide the initial electricity needed to start other power plants.
2. Voltage and Frequency Support: CAES can provide stable power to help re-establish proper frequency and voltage levels in the grid, smoothing the restart process.
3. Critical Load Support: During outages, CAES can supply electricity to essential services (e.g., hospitals, water treatment, data centers), reducing the severity of disruptions.
Additionally, CAES facilities contribute to overall grid stability by offering services such as:
• Frequency Regulation: Adjusting output quickly to match demand fluctuations, helping maintain frequency within acceptable ranges.
• Voltage Support: Operating compressors and turbines in a way that provides or absorbs reactive power.
• Spinning and Non-Spinning Reserves: Standing ready to inject power on short notice to counter unexpected demand spikes or generator failures.
This combination of black start capability and ancillary services strengthens the reliability and resilience of modern power grids, making CAES an attractive option for grid operators seeking to manage high penetrations of renewable energy while maintaining system stability.
12. Applications of CAES
12.1 CAES in Data Centers
Data centers are critical facilities with constant, high power demands. As global internet usage and cloud services expand, data centers are projected to consume an ever-increasing share of global electricity. CAES can help data centers achieve sustainability goals and manage power costs:
• Peak Shaving: Data centers can purchase grid power during off-peak, lower-cost periods to compress air and store energy, then use it during peak demand, saving on electricity bills.
• Backup Power: CAES can provide reliable backup power during grid outages, functioning similarly to diesel generators but with fewer emissions.
• Renewable Integration: By storing excess wind or solar power, CAES allows data centers to maximize renewable energy usage, aligning with corporate sustainability targets.
• Challenges: Traditional CAES requires significant space (especially for underground caverns), and the round-trip efficiency remains lower than certain battery systems. However, advanced CAES designs and above-ground solutions (e.g., high-pressure tanks) are under development, potentially expanding data center integration options.
Though there are no widely publicized, fully commercial case studies of data centers relying on CAES today, interest in this combination continues to grow as data centers explore alternatives to diesel generators and large battery banks.
12.2 CAES in Eco-Resorts
Eco-resorts aim to minimize environmental impact while offering upscale tourist experiences. Integrating CAES can support sustainability initiatives by providing:
• Off-Grid Energy Storage: In remote locations with ample renewable resources but unreliable grids, CAES can store surplus solar or wind energy for use during peak demand, reducing reliance on diesel generators.
• Long-Duration Storage: Eco-resorts often require consistent power for lighting, HVAC, and guest services. CAES can bridge multi-hour or overnight gaps in renewable generation.
• Reduced Carbon Footprint: Adiabatic CAES can eliminate fuel combustion, preserving the pristine environment that eco-resorts depend upon.
• Educational Opportunities: Showcasing CAES technology can be an attractive feature for eco-conscious guests, aligning with the resort’s green branding.
While direct examples of eco-resorts implementing CAES have not been widely documented, the compatibility of CAES with sustainable tourism goals is clear, especially as smaller-scale CAES technologies mature.
12.3 CAES for Smart Cities
Smart cities prioritize efficient resource management, digital connectivity, and sustainability. CAES fits neatly into these objectives by:
• Supporting Renewable Grids: Large-scale energy storage can stabilize grids with high solar or wind penetration, ensuring reliable power across urban areas.
• Demand Response and Peak Shaving: In a smart city, real-time data on energy consumption can guide the optimal charging and discharging of CAES, reducing peak loads.
• Disaster Resilience: CAES can provide backup power for critical city infrastructure—traffic lights, hospitals, water treatment—during emergencies.
• Integration with AI Systems: Smart grids increasingly rely on AI for predictive analytics, demand forecasting, and real-time optimization. CAES can interface with these systems to dispatch stored energy in the most efficient manner.
The Hubei Yingchang project in China offers a glimpse into large-scale CAES integration with smart grid ambitions. By providing hundreds of megawatts of flexible capacity, the system helps incorporate more renewable generation into the local grid and supports China’s broader movement toward a smart, low-carbon energy infrastructure.
13. AI Integration in CAES
Artificial Intelligence (AI) is transforming how modern energy systems operate, and CAES is no exception. AI-driven analytics, machine learning, and advanced control algorithms can dramatically improve the design, efficiency, and reliability of CAES installations:
13.1 AI for System Modeling and Optimization
• Digital Twins: AI allows the creation of “digital twins”—virtual replicas of CAES systems used for simulation and real-time monitoring. Engineers can test operational strategies, optimize design parameters, and predict system performance under various conditions without risking real-world equipment.
• Design Optimization: Machine learning algorithms can process vast datasets to identify the most efficient system configurations, from compression ratios to thermal energy storage design. This approach leads to faster, more robust improvements compared to traditional trial-and-error engineering.
13.2 AI-Driven Predictive Maintenance
• Condition Monitoring: Sensors integrated into turbines, compressors, and heat exchangers collect operational data in real time. AI algorithms detect anomalies, indicating wear or impending failures.
• Maintenance Scheduling: By anticipating failures before they occur, operators can plan maintenance proactively, reducing downtime and extending equipment lifespan. Predictive maintenance can cut unplanned outages by up to 50% and optimize replacement cycles for expensive components.
13.3 AI for Renewable Integration and Grid Management
• Demand and Generation Forecasting: AI excels at forecasting both energy demand (e.g., daily load curves) and renewable generation (wind patterns, solar irradiance). Accurate predictions enable CAES systems to charge and discharge optimally.
• Market Participation: In deregulated markets, real-time electricity prices can vary significantly. AI algorithms help CAES operators decide when to buy power for compression and when to sell power for profit, maximizing economic returns.
• Efficiency Improvements: Some startups claim AI-driven thermal management can boost CAES system efficiency by 10-15% while reducing overall costs. By controlling the timing and rate of heat transfer, AI can minimize exergy loss and optimize round-trip efficiency.
As AI adoption expands, we can expect more advanced hybrid approaches that combine CAES with other storage or generation technologies, orchestrating complex multi-faceted power systems. The result could be near-seamless integration of intermittent renewables, minimized curtailment, and more stable, resilient grids. While large-scale commercial adoption of AI-enabled CAES remains in its early stages, ongoing pilot projects and research initiatives highlight the transformative potential of these combined technologies for future energy systems.
14. Future Prospects and Research Directions
CAES technology is poised to play a growing role in global energy transitions, especially as countries and regions commit to higher shares of renewables and stricter emissions targets. Several areas of ongoing research and development could define the trajectory of CAES in the coming decades:
1. Advanced Adiabatic Systems: Achieving cost-effective, reliable, and high-temperature thermal energy storage is a primary research goal. Materials scientists and thermal engineers are exploring phase-change materials, molten salts, and ceramic composites that can store and release heat efficiently with minimal degradation over repeated cycles.
2. Hybrid Configurations: Combining CAES with other technologies—such as biomass, combined heat and power (CHP), fuel cells, or Organic Rankine Cycles (ORCs)—could further improve overall system efficiency. In some pilot projects, waste heat from industrial processes or gas turbines is captured and utilized within CAES cycles, creating synergistic efficiencies.
3. Above-Ground CAES: For regions lacking suitable geology, researchers are investigating advanced high-pressure tanks and modular systems. These can be scaled up or down depending on local energy demands. Materials innovation (e.g., in carbon-fiber composites) could reduce costs and weight, making above-ground CAES more practical.
4. Small-Scale and Distributed CAES: Beyond large utility projects, smaller-scale CAES may find applications in remote microgrids, farms, or eco-resorts. As the technology matures, smaller units—potentially integrated with solar panels or small wind turbines—could offer an alternative to batteries for off-grid or near-grid energy storage.
5. AI-Driven Optimization: The integration of AI in system design, real-time control, maintenance scheduling, and grid coordination is a major area of focus. Deep reinforcement learning algorithms, for instance, can optimize charging and discharging in near-real-time, responding dynamically to fluctuations in renewable generation and market prices.
6. Regulatory Frameworks and Standards: A supportive policy environment can accelerate the deployment of CAES. The establishment of standardized performance metrics (e.g., round-trip efficiency, emissions, cost per kWh), safety protocols, and streamlined permitting for underground storage could reduce uncertainties for project developers.
7. Decarbonization Goals: As governments and corporations commit to net-zero or low-carbon goals, the demand for long-duration energy storage solutions that can balance intermittent renewables is expected to rise. CAES fits well into scenarios requiring multi-gigawatt-hour scale capacity with high discharge durations.
Overall, CAES stands at an exciting intersection of advanced materials research, energy market evolution, and the pressing global need for sustainable storage solutions. Continued progress in adiabatic designs, improved cost structures, and alignment with AI-enabled smart grids could make CAES a cornerstone technology in the evolving clean energy landscape.
15. Conclusions
Compressed Air Energy Storage (CAES) represents a versatile and powerful technology that addresses many of the challenges associated with integrating large amounts of renewable energy into modern power grids. By leveraging periods of surplus electricity to compress air and then harnessing that stored energy during peak demand, CAES effectively smooths out the intermittent nature of wind and solar power.
In its most developed commercial forms—such as diabatic systems at Huntorf (Germany) and McIntosh (United States)—CAES has provided decades of reliable service. Ongoing innovations, particularly in adiabatic (A-CAES) and near-isothermal designs, aim to boost the round-trip efficiency beyond 70%, minimizing or even eliminating reliance on fossil fuels for reheating. This makes CAES increasingly attractive in decarbonized energy scenarios.
The technology’s advantages include long-duration storage, large capacity, cost-effectiveness at scale, and the ability to provide critical grid services like black start capability, frequency regulation, and peak shaving. At the same time, CAES faces challenges: site selection often depends on geological suitability, and thermodynamic management of heat remains complex. However, examples such as the Hubei Yingchang project in China show that with innovative planning—e.g., reusing abandoned salt mines—developers can address both geological and environmental constraints while significantly contributing to regional decarbonization and grid reliability.
Looking ahead, the integration of AI holds particular promise for CAES, enabling advanced system modeling, predictive maintenance, real-time optimization, and seamless coordination with other energy assets. These AI-driven enhancements can improve efficiency, reduce costs, and ensure that CAES facilities operate optimally within dynamic and increasingly renewable-dominated grids.
Applications beyond typical utility-scale storage are also emerging. Data centers, facing immense power demands and seeking to reduce carbon footprints, are investigating CAES as a large-scale alternative or supplement to batteries. Eco-resorts and remote sites may also benefit from smaller CAES systems that store locally generated renewable energy. In smart cities, CAES can serve as a backbone for robust, resilient energy systems capable of handling complex load patterns and supporting diverse end uses.
In sum, CAES remains a cornerstone technology for tackling the grand challenge of large-scale, long-duration energy storage in a low-carbon future. Continued research, policy support, and technological breakthroughs in thermal management and AI will help CAES fulfill its considerable potential and accelerate the global transition to sustainable, reliable energy systems.
Final Thoughts
If you want to talk over your options, click here to schedule a time to chat with our Principal Joe MacDonald see how we can help you revolutionize global project development, management, and delivery
If you enjoyed The Sustainable Architect edition, I have a quick favour to ask:
1. Subscribe to The Net Zero Strategist the button at the top of this post so you don’t miss a single #urbanao post.
2. Share this post with your network on LinkedIn if you think they could benefit from the message using the Share button below.